Science
Related: About this forumCO2 Hydration Conditions during Hydrogen Evolution and Carbon Dioxide Reduction Gas Diffusion Electrode Reactions
The paper to which I'll refer is this one: Is Hydroxide Just Hydroxide? Unidentical CO2 Hydration Conditions during Hydrogen Evolution and Carbon Dioxide Reduction in Zero-Gap Gas Diffusion Electrode Reactors Henrik Haspel and Jorge Gascon ACS Applied Energy Materials 2021 4 (8), 8506-8516.
As I often note, electricity is a thermodynamically degraded form of energy, and, as such, despite the common public misapprehension that it is "green," perhaps driven by familiarity and a large dollop of wishful thinking, it is dirty. The relationship between thermodynamics and environmental cost is more or less direct; any system with poor thermodynamics will require the generation of more energy than necessary. The generation of electricity wastes energy, even without further waste by the storage of electricity in batteries, or worse, hydrogen.
Electricity production on this planet, as is the case with all forms of energy, remains increasingly dependent on combustion, overwhelmingly the combustion of dangerous fossil fuels.
It follows that the electrochemical reduction of CO2 using electricity, just like the electrochemical reduction of water to make hydrogen will be intrinsically involved with exergy destruction, that is, poor thermodynamics.
There is an important caveat to the above statement; electricity can represent a thermodynamically improvement where it is a side product which recovers exergy by the transformation of waste heat.
Consider the Sulfur Iodine Cycle, a process for the thermochemical decomposition of water into hydrogen and oxygen without the use of thermodynamically degraded electricity. In this process, H2SO4, sulfuric acid is decomposed into SO2 gas and O2 gas and steam H2O at high temperatures, on the order of 1000°C. Some of this heat can be recovered for another reaction in the cycle, the decomposition of hydrogen iodide (HI) gas into H2 and I2 gases, but this step takes place at a much lower temperature than the decomposition of hydrogen iodide gas. In addition, at high temperatures, the products of sulfuric acid decomposition can recombine to result in the reverse reaction, the formation of sulfuric acid, to take place.
Thus it is useful to consider cooling the mixture SO2 gas and O2 gas and steam H2O rapidly to a temperature at which SO2 gas. The critical temperature, the highest temperature at which a gas can be liquified, of SO2, Tc gas is 430.3K, or 147.1°C, well above the boiling point of water, suggesting one could recover exergy by placing a steam engine in the stream. This case is a simplification, and for various reasons there are better approaches for exergy recovery from the sulfur iodine cycle, but it is illustrative. The point is that in producing chemical energy, that represented by hydrogen, which can be used for the synthesis of portable fuels, one can recover electricity as a side product, rather than rejecting the heat to the atmosphere.
I do not expect energy sanity to emerge in the future, since popular thinking is either reactionary or conservative but I do concede that energy sanity is feasible, if not likely.
The paper referenced at the outset of this post is about the electrochemical reduction of carbon dioxide, a much studied concept. The issue of carbon dioxide utilization can often be subject to appeal by people trying to greenwash fossil fuels, as is the case with people trying to rebrand fossil fuels as hydrogen, here at DU and elsewhere. Nonetheless, although the thermodynamics of the reduction of carbon dioxide requires that we not only produce all of the energy that put it there, the enthalpy, we must also produce additional energy to overcome the entropy of mixing. I make this point repeatedly. Often, as is the case with people selling fossil fuels by attempting to rebrand them as "hydrogen" this sort of thing, similar to the nonviable and unpracticed scam of carbon dioxide sequestration, appeal to these ideas is to greenwash fossil fuels. Nevertheless, so far as direct air capture, or better, capture via the intermediate of seawater, is concerned, I think it is important, to use the cliche, "not to throw the baby out with with the bathwater."
The question to my mind with respect to direct air (or seawater) capture and reduction (utilization) is "Do we have any choice but to do otherwise?"
For direct air capture, I often consider strong bases, among the strongest being the aqueous hydroxides of rubidium and cesium, both of which are fission products, with cesium having the additional benefit of being highly radioactive, and thus capable of mineralizing extremely difficult to destroy pollutants, notably those having carbon fluorine bonds.
Thus, considering the feasibility of the case - not likely, but feasible - where electricity is a side product rather than the main product of the production of heat, the paper caught my eye, as I was poking around for the kinetics of the hydration of carbon dioxide.
From the introduction:
All the three basic CO2RR flow cell configurations, namely, (1) zero-gap or membrane electrode assembly (MEA)-type, (2) three-compartment liquid catholyte type, and (3) microfluidic electrolyzers, have their own advantages and disadvantages. (4,5,11) Despite the well-defined cathode environment in a liquid catholyte cell, achieving high energy efficiency is hindered by the relatively high Ohmic resistance of the cell. Moreover, stable cell operation needs continuous control of the pressure difference between the CO2 stream and the liquid cathode compartment. By pressing the cathode and anode GDEs directly against the AEM, an MEA is formed. (12) The main advantage of a gas-fed MEA-type or zero-gap electrolyzer is the lower operating cell voltage due to the absence of a catholyte stream and the corresponding lower Ohmic losses. (11,13) However, this poses a further challenge in developing a molecular-level understanding of the ongoing processes due to the ill-defined local environment.
On the other hand, anionic CO2 electroreduction products can cross AEMs (14) along with a substantial CO2 loss in the form of HCO3 and CO32 as CO2 reacts with OH ions either generated in CO2RR or already present in the electrolyte. (15) An AEM acts as a CO2 pump; one or two CO2 molecules are liberated at the anode when carbonate or bicarbonate ions pass through the membrane, respectively. (15) The so-called carbonation process, that is, the coexistence of OH, HCO3, and CO32, is a present challenge to be solved in AEM fuel cells. (16,17) In flow cell CO2 electrolysis, carbonation is the main source of the low CO2 utilization efficiency. (18) Up to 70% of the reactant feed is captured under basic conditions and later released in the form of gaseous CO2 at the anode. (19−21) Moreover, carbonate/bicarbonate salt precipitates at the cathode in alkaline electrolyzers, resulting in activity loss and long-term stability issues in high current electrolysis. (22,23)
Herein, we aim to elucidate the charge transport through the AEM in a zero-gap flow electrolyzer in 2e processes. To this end, we present a detailed description of the mass balance for CO2-to-CO and hydrogen evolution reaction (HER) up to 900 mA cm2 on carbon-supported silver (Ag/C) and various non-CO2RR (Pt/C, IrO2/C, and NiFeCo/Ni) GDEs using KOH and KHCO3 anolytes. We recorded the reactant flow rate depression, the O2 and CO2 evolution at the anode, and the anolyte pH change during electrolysis. The dominant charge carriers were identified at a wide range of operational parameters (current density, CO2 feed rate and partial pressure, and different anolytes and catalysts), and a variation in the chemical nature of the transported ionic species was found. This in turn implies differences in the non-electrochemical CO2 consumption at the cathode/membrane interface through unidentical CO2 hydration conditions in MEA-type zero-gap electrolyzers...
Two figures from the paper:
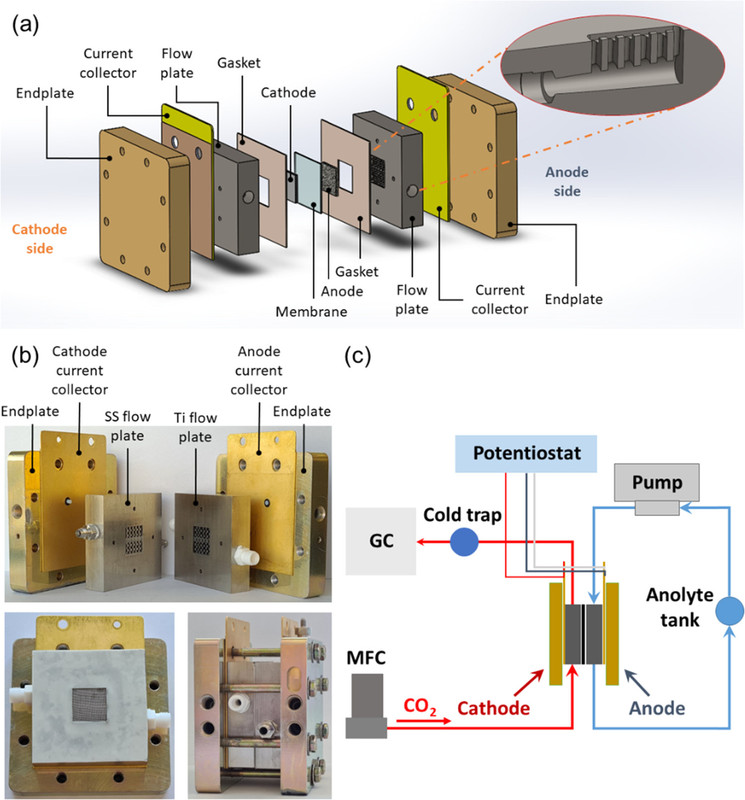
The caption:
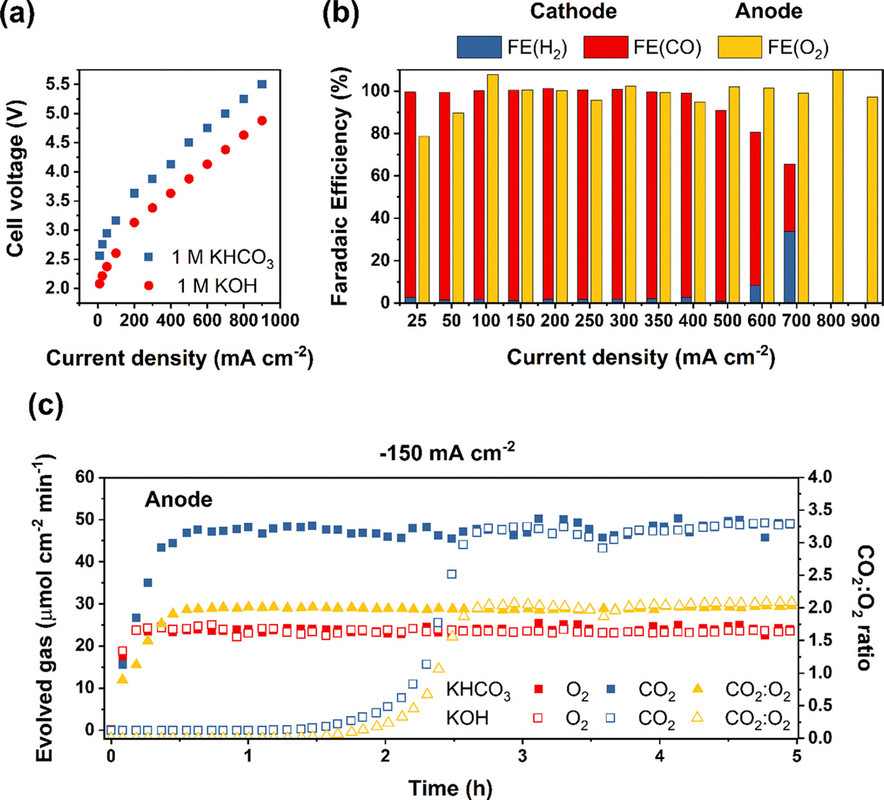
The caption:
It is important to note that what is being discussed is Faradaic efficiency as opposed to Thermodynamic efficiency, which is much lower. The former refers to the utilization of electrons to generate the product, the latter to the waste of energy that the process involves, that is exergy destruction. (In electrochemical papers this is usually discussed in terms of overvoltage, the difference between the voltage required by the Nerst equation and that required for the reaction to actually take place in the real world, that is theoretical vs. observed.)
The mixture of CO and hydrogen is known as "syn gas" from which any important commodity currently obtained from dangerous petroleum can be synthesized, along with portable fuels vastly superior to say, gasoline and/or diesel fuel and/or kerosene (jet fuel).
Have a nice Sunday afternoon.